Is cancer a disease of abnormal cellular metabolism?
- Greg Rauscher
- Oct 31, 2024
- 9 min read
Updated: Jan 26
NIH Article
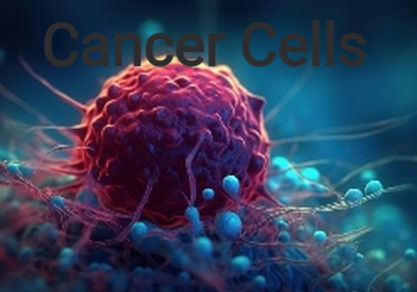
In the 1920s, Otto Warburg observed that tumor cells consume a large amount of glucose, much more than normal cells, and convert most of it to lactic acid. This phenomenon, now known as the ‘Warburg effect,’ is the foundation of one of the earliest general concepts of cancer: that a fundamental disturbance of cellular metabolic activity is at the root of tumor formation and growth. In the ensuing decades, as it became apparent that abnormalities in chromosomes and eventually individual genes caused cancer, the ‘metabolic’ model of cancer lost a good deal of its appeal, even as emerging technologies were exploiting the Warburg effect clinically to detect tumors in vivo. We now know that tumor suppressors and proto-oncogenes influence metabolism, and that mutations in these genes can promote a metabolic phenotype supporting cell growth and proliferation. Thus, these advances have unified aspects of the metabolic and genetic models of cancer and have stimulated a renewed interest in the role of cellular metabolism in tumorigenesis. This review reappraises the notion that dysregulated cellular metabolism is a key feature of cancer and discusses some metabolic issues that have escaped scrutiny over the years and now deserve closer attention.
The Warburg effect, also known as aerobic glycolysis, is defined as a high rate of glucose (Sugar energy) utilization and lactate production despite the presence of sufficient oxygen to oxidize glucose carbon in the mitochondria. Recognition of this unusual metabolic phenomenon stems from experiments performed by the German physiologist Otto Warburg, starting in the 1920s. In those experiments, Warburg compared the metabolism of rapidly proliferating mouse ascites tumor cells to that of differentiated, quiescent cells from organs of the adult animal. He proposed that a fundamental impairment of cellular respiratory capacity was the root cause of all cancer, a bold and controversial claim that was ultimately rejected despite his continued writing and lecturing on the subject for some 40 years. Nevertheless, appreciation of the Warburg effect as a feature of tumor cell metabolism has survived its namesake by a long stretch. Today, the glycolytic activity of tumors is not only accepted, but exploited clinically by F-deoxyglucose positron emission tomography (FDG-PET), which detects tumors precisely by virtue of their enhanced ability to take up and metabolize glucose compared to normal tissue. The Warburg effect remains the most frequently cited evidence that tumors display dysfunctional metabolism.
Recently, interest in tumor metabolism has enjoyed a renaissance as an ever-growing number of reports uncovers the molecular connections between transformation and cell metabolism, and as technological improvements increase the feasibility of studying tumor metabolism in vivo. The field seems poised to offer significant insights into tumor biology over the next decade. As such, it is worth re-examining the evidence for a bona fide connection between altered cellular metabolic state and tumorigenesis: does the notion of such a connection stand up to our current understanding of tumor biology and cancer genetics? If there is such a link, then the following should be true:
1. Tumor cells should have metabolic activities that differ from non-transformed, quiescent cells, and these activities should be required for tumor growth;
2. The mutations in tumor suppressors and proto-oncogenes that promote cancer should regulate the metabolic activities observed in tumors; and
3. Mutations in metabolic enzymes should, in at least some cases, promote tumorigenesis.
DO TUMOR CELLS HAVE METABOLIC ACTIVITIES THAT ARE DIFFERENT FROM QUIESCENT CELLS AND ARE REQUIRED FOR TUMOR GROWTH? Cancer & Metabolism
Most studies on tumor metabolism have been motivated by one of two general concepts about the way cell metabolism is regulated. The first is that tumor metabolism is primarily a response to stresses imposed upon cells during tumor growth. There is abundant evidence that some stresses, particularly hypoxia, exist in the tumor microenvironment and exert effects on metabolism3–5. But the high glycolytic flux in tumors can appear even when oxygen is abundant, and the metabolic consequences of hypoxia include specific impairments of protein and lipid synthesis that are counterproductive to cell growth and proliferation6–8. These observations suggest that the cellular responses to tumor hypoxia, including enhanced glycolysis, serve to facilitate tumor cell survival, not growth. This is also true in non-transformed cells, which rely on glycolysis to survive periods of hypoxia9. When oxygen delivery improves and rapid cell growth resumes, the persistence of glycolysis is likely due to other factors.
Alternatively, one can presume that tumor cell metabolic activities function primarily to support the unusually high rates of cell growth and proliferation found in tumors. Since each round of replicative cell division requires a doubling of protein, lipids and nucleic acids, it stands to reason that tumor cell metabolism must provide the energy and biosynthesis needed to meet this challenge. Rapid tumor growth requires the ability to capture nutrients and process them in the appropriate metabolic pathways to convert their carbon and nitrogen into macromolecules. All of the activities discussed in this section (the Warburg effect, fatty acid synthesis and mitochondrial glutamine metabolism) occur during cell growth, and evidence suggests that the three pathways cooperate in such a way as to maximize the production of macromolecules in proliferating cells.
Glutamine metabolism is second only to the Warburg effect in terms of historical significance to the study of tumor cell metabolism. Glutamine is the most abundant amino acid in human plasma and participates in many metabolic pathways required for normal cell function. In addition to its role in protein synthesis, it provides nitrogen for the synthesis of nonessential amino acids, purines, pyrimidines and hexosamines, and is the major source of glutamate used for glutathione synthesis. Tumor cells have long been known to consume glutamine at high rates in vivo and to require high concentrations of glutamine to survive and proliferate in vitro. Classical studies on tumor cell metabolism in culture demonstrated that glutamine is an important carbon source since most of the glutamine consumed is used as a respiratory substrate in the mitochondria rather than for protein synthesis31. More recent experiments have demonstrated that suppressing mitochondrial glutamine metabolism can alter gene expression, accelerate apoptosis and stimulate cellular differentiation. Therefore, glutamine metabolism has the potential to integrate a large number of cellular activities that support tumorigenesis.
WHERE DO WE GO FROM HERE?
At present, the data on tumor cell metabolism make a compelling argument that during the process of transformation, cells acquire a fairly stereotyped set of metabolic characteristics that enable them to grow and proliferate with reduced dependence on extracellular signals, and that the mutations in tumor suppressors and proto-oncogenes that cause cancer contribute to this ‘metabolic transformation.’ There are a number of important questions that still need to be addressed before we can appreciate fully the role of metabolism in the development, progression, and treatment of cancer. Below are six areas that are in particular need of attention.
First, there is still a large gap between our understanding of tumor cell metabolism in vitro and tumor metabolism in vivo. The inhibitor and RNA interference experiments cited above demonstrate the requirement of certain enzymes for tumorigenesis, but the ability to observe the metabolism of live tumors would provide a much richer understanding of the biochemical aspects of tumor growth and would support more rigorous examination of the effect of tumorigenic mutations on metabolic flux. The use of 18FDG-PET and conventional NMR spectroscopy have provided some validation of the Warburg effect and other pathways in live tumors but are currently best suited to give snapshots of metabolism rather than robust measurements of metabolic flux. The application of more sensitive methods such as the use of probes labeled with hyperpolarized 13C may ultimately support flux analysis in vivo.
Second, the regulation of anaplerosis and of glutamine metabolism in general has not been carefully studied with respect to cell signaling. While glutamine consumption is a general metabolic feature of cell proliferation, it is unknown whether all tumor cells must use glutamine as the major source of carbon for anaplerosis. In a few cases, cellular biosynthesis and growth have been correlated with the induction of alternative anaplerotic pathways that do not involve glutamine metabolism, and thus the universality of glutamine-based anaplerosis is debatable. Furthermore, while stimulation with mitogens can enhance glutamine utilization in various cell types, neither the signaling pathways responsible for this activity nor the specific pathways of glutamine metabolism (e.g. glutaminolysis vs. glutamine-based anaplerosis) have been characterized. Resolving these issues will go a long way towards integrating our understanding of cell signaling and the metabolism of cell growth. Considering that glucose and glutamine play complementary roles in proliferating tumor cells, it will be interesting to test whether they are co-regulated by the same signaling pathways and affected by the same tumorigenic mutations.
Third, many studies have sought to unravel the complex relationships between oxidative stress, cellular transformation and cancer. At the level of cell metabolism, there are many open questions about how NADPH-producing systems are regulated and whether these mechanisms are altered in tumor cells. Rapidly proliferating tumor cells require NADPH both to maintain pools of reduced glutathione and to support the reductive biosynthetic reactions needed for anabolic metabolism. The two NADPH-producing systems discussed above, G6PD and cytosolic malic enzyme, are expressed in tumors of various histological types, and in some cases their activities are almost equal in proliferating tumor cells, implying equivalent contributions to the NADPH pool. It is interesting that G6PD deficiency, a common X-linked condition, does not alter the risk of death from cancer. This suggests that malic enzyme or other systems can provide sufficient NADPH to support tumorigenesis in G6PD-deficient cells, and that selective inhibition of malic enzyme in individuals with G6PD deficiency might suppress tumor growth. Conversely, maintaining an NADPH pool and a robust response against oxidative stress is critical in preventing tumorigenesis, as emphasized by recent studies on the transcription factor NF-E2-related factor-2 (Nrf2). Nrf2 is sequestered in the cytosol through association with its repressor Keap1 (Kelch ECH associating protein 1).
Exposure to oxidative and other stresses allows Nrf2 to translocate to the nucleus, where it heterodimerizes with small Maf-family proteins and binds promoters containing antioxidant response elements, thus inducing expression of genes that serve antioxidant functions. This protects cells from apoptosis during exposure to oxidizing agents, UV irradiation and other stresses. Knockout mice lacking Nrf2 have enhanced susceptibility to cancer when exposed to chemical mutagens. Future studies will be aimed at exploiting the Nrf2 pathway as a strategy to prevent cancer, and at understanding other mechanisms by which normal cells and tumor cells respond to oxidative stress.
Fourth, almost all previous studies on tumor cell metabolism have been performed in unsynchronized populations of cells distributed throughout the cell cycle, so that the resulting data represent average cell metabolic activity throughout the cycle. The temptation is to assign the greatest biological importance to the pathways that appear to be the most active, but such an interpretation could miss the significance of pathways that are transiently induced at specific, critical points of the cell cycle. One can envision two ways to identify these activities: by performing flux analysis in synchronized populations of cells or by using inhibitors and genetic models to determine the effect of disrupting candidate metabolic activities on progression through the cell cycle. There is already strong evidence that interfering with metabolism profoundly affects the cell cycle. In fibroblasts, glucose deprivation imposes an AMPK- and p53-dependent G1/S phase arrest, and in Drosophila, elimination of ETC components stimulates several distinct signaling pathways that culminate in arrest at the G1/S transition. These efforts are relevant to metabolically directed cancer therapy, especially if such therapies are used as adjuvants to existing strategies that already exert cell cycle-specific effects. For example, if ionizing radiation is most effective on cells at the G2/M transition, then a metabolic therapy targeting a G2-specific activity might increase the fraction of cells at that stage, thereby increasing the overall efficacy of treatment.
Fifth, it is possible that normal but genetically defined variation in the expression of metabolic enzymes could influence cancer risk and progression. Microarray analysis of gene expression in cells from healthy individuals has shown that some metabolic enzymes and transporters are highly variable in their mRNA abundance, and that the variance is defined genetically. Currently, the association between disease states and variance of expression in these genes is unknown. But the abundance of certain metabolic enzymes in tumors is correlated with poor clinical outcome in various forms of cancer. If an individual’s cells have an unusually high expression of a key enzyme(s) at baseline, then the acquisition of a transforming mutation in those cells might translate into enhanced growth and clinically aggressive features of the resulting tumor. Since the variance in expression of the enzyme would not independently cause cancer, the genes responsible for the variance would probably not be identified in linkage studies to find cancer-causing genes.
Finally, it will be interesting and important to fit tumor metabolism back into the context of the entire cancer patient. Regardless of the increased metabolic autonomy of tumor cells, tumor growth still relies on the metabolism of the host both to provide nutrients and to remove secreted waste products. Yet the issue of how tumors influence whole-body metabolism is still very much an open question, and one that relates directly to the health of cancer patients. For example, the phenomenon of cancer cachexia has been appreciated for centuries, but its causes are only partially understood and include processes that raise whole-body energy expenditure and stimulate catabolism in both the fat and muscle. Does the catabolic response function in part to provide nutrients to the tumor? In rats, tumor growth was associated with progressive increases in the synthesis and release of glutamine from muscle, ultimately resulting in decreased glutamine stores in the face of relentless tumor growth, consistent with glutamine ‘steal’ by the tumor. Other studies have demonstrated markedly enhanced Cori cycle metabolism to convert lactate back to glucose in the liver of cancer patients who were suffering from progressive weight loss. These studies demonstrate the interconnected nature of whole-body metabolism in patients with cancer. They also underscore the fact that any hope for rational, metabolically directed cancer therapies depend on the development of a comprehensive understanding of metabolism in the host as well as in the tumor.
Comments